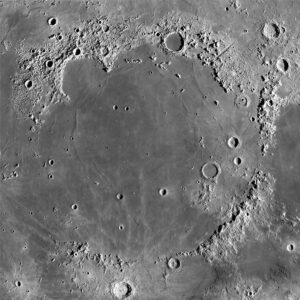
Martian stratigraphy – lessons from the Moon
The universality of fundamental laws is firmly embedded in the scientific milieu; viz. the laws of thermodynamics, gravity, electromagnetism. The importance of this condition, both philosophically and practically, is that our conduct of science is admissible even when we step beyond the bounds of terra firma. The fundamental principles, or propositions, that guide stratigraphic analysis on Earth are founded on these laws (e.g., the conservation of energy, entropy). This is a fortunate circumstance because it gives us a warrant to use these principles on other planets. Foremost are Nicholas Steno’s propositions that articulate stratigraphic superposition; propositions that allow us to infer the time and space relationships among bodies of rock, that are fundamental to geological mapping and unraveling of stratigraphic architecture.
Martian stratigraphy and geological mapping are based on the principles of rock-body superposition. But unlike Earth, they are based entirely on remotely observed superposition of geomorphic entities – primarily impact craters and their relationship with geological map units. These observations were derived from successive generations of satellite imagery, augmented by more “hands on” data from surface rovers, beginning with Sojourner in 1997. Impact craters are seen as events in time (the actual meteorite-asteroid-comet impacts) as well as geomorphic structures. Superposition involves both space and time; it recognizes younger craters on older craters.
Like Earth-bound stratigraphic schemes, Martian nomenclature is based on a 3-fold stratigraphic subdivision:
- Rock-stratigraphic (or lithostratigraphic) units: rock and geomorphic units.
- Time-stratigraphic (time-rock) units: based on the relative ages of broad lithostratigraphic units.
- Chronostratigraphic (time) units: based on the ages of time-stratigraphic units. Actual ages are provided by radiometric dating.
The starting point is the Moon
The theoretical basis for establishing planetary stratigraphic schemes based on the superposition of impact craters and crater densities was first developed for the Moon. The Moon’s impactors originated from regions of the solar system traversed by all the rocky planets, and it is reasonable to assume that impact frequencies were similar for each of those planets. This is the principle wherein the methodology established for the Moon can be applied to the rocky planets.
Craters size frequency distributions
Dating of rock bodies is inevitably relative until such time that radiometric ages are available. This was the case for lunar mapping until the Apollo program returned rock samples (1969-1972). Conditions and assumptions that permit the relative dating of craters and clusters of craters are:
- The location of craters is random across the lunar surface. Thus, for a specified time period, crater flux would have been consistent across the entire lunar surface.
- Crater density is related to geological age. Older lunar surfaces will be more cratered than younger surfaces.
- Distinguishing primary craters (i.e. direct impacts) from secondary craters that form from material ejected by the primary impact.
- Distinguishing endogenic craters from impact craters – i.e. those formed by volcanic eruptions or gravitational collapse (e.g., calderas).
- Older craters will be more degraded than younger, fresher craters. On the moon, crater degradation occurs when there is direct overlap by younger craters and their ejecta, and from gravitational collapse of crater walls. Masking of primary and secondary craters can also occur on lunar mare where they have been inundated by flood basalts.
There is a measurable relationship between crater size and crater frequency for each surface map unit, referred to as the Crater Size Frequency Distribution (CSFD, or SFD) (Tanaka, 1986; Hartmann and Neukum, 2001; Tanaka and Hartmann, 2012). Each map unit should be morphologically homogeneous (lunar mare are obvious examples of morphological homogeneity). Morphological homogeneity is determined from:
- Consistent geomorphic features such as rugged highlands, basins (very large craters), or mare (basalt lava flood plains).
- Clusters of craters having a similar degree of preservation.
- Albedo.
- Identifiable map boundaries (mappability), and
- Regional cross-cutting structures such as lava flow boundaries, or the walls of large craters.
The basic representation of a crater SFD for geomorphic (map) units like mare, basins, and highlands is a log-scale plot of cumulative crater frequency (the number of craters per km2) against crater diameter. Data is generated by counting morphologically similar craters of a certain diameter. Log-scales are used because crater sizes range over 5 or 6 orders of magnitude, although a linear scale is sometimes used for craters where there is a restricted range of diameters. Thus, the number on the frequency axis is an order-of-magnitude, and not an absolute number. Small craters tend to be more numerous than larger craters. Therefore, to avoid sample bias towards small craters, the analysis involves counting the number of craters greater than a certain diameter for a designated area.
The example below shows the cumulative frequency curves for craters with diameters greater than 20 km in three lunar mare; each mare has relatively well defined map boundaries. The number of craters for Mare Nectaris is significantly higher than for Mare Imbrium and on this basis is considered relatively older. Likewise, Mare Oriental would be the youngest of the three.
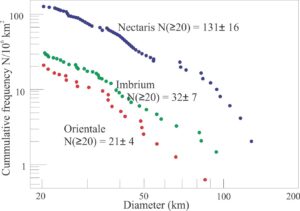
This analytical method has been conducted for map units over most of the Moon’s surface. The method is analogous to the use of fossils to develop a relative rock-stratigraphic framework on Earth. The resulting lunar framework identifies five main rock-stratigraphic units at the System rank; the equivalent stratigraphic rank on Earth includes Jurassic, Triassic etc. The earliest attempts at developing this framework used Earth-bound telescope images to map very large morphological features such as the mare and large basins. The advent of satellite imagery has allowed refinement of this framework.
From rock-stratigraphy to chronostratigraphy
The second step in developing a lunar stratigraphy uses SFDs to calculate ‘model’ ages for the rock-stratigraphic units. For details of the mathematics involved see Wilhelms et al., 1987; Ivanov, 2001, Hartmann, and Neukum, 2001, op cit; Tanaka and Hartmann, 2012, op cit. The SFDs create a picture of the relationship between the size of craters and the number of craters counted. This can be expressed as a polynomial – the crater production function (CPF). Some of the first CPFs were developed for lunar mare because they are relatively smooth, easily mapped, and craters are relatively fresh. The next step is to use the CPFs to develop a lunar chronology function that expresses the cumulative frequency of craters equal to or greater than a specified diameter against age – this function is calibrated using radiometric dates from lunar samples.
A pivotal step in developing the Lunar stratigraphic framework has been calibration of chronostratigraphic boundaries using radiometric dates of samples returned from the Apollo missions (1969-1972), the Soviet Union’s Luna (unmanned) program (1970-1976), and the (unmanned) Chang’e 5 (2020) mission. The ages range from about 4.46 to 3.16 Ga. Chang’e 5 basalt samples from Mare Oceanus Procellarum are in the range 1.97-2.03 Ga, the youngest lava crystallization dates so far for lunar samples (Li et al., 2021, OA). The ages, when applied to the CPFs, contribute to the refinement of time limits for lunar rock-stratigraphic units. The resulting chronostratigraphic units have the rank of Period.
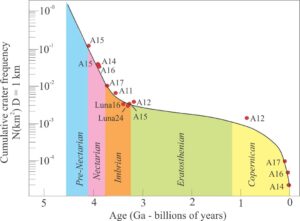
Modification of this basic scheme has been proposed by Guo et al., 2023, based on image mapping of crater ejecta and mare-filling by lava for the large South Pole-Aitken Basin. They replace the Pre-Nectarian with a magma ocean period following planetary accretion, subdivide the Nectarian, and re-evaluate chronostratigraphic ages for the other major periods.
Martian stratigraphic framework
The geological periods currently used in Martian stratigraphy were derived using the same processes and rules used for the Moon – superposition of geological map units, crater densities, and fitting the production functions and chronology functions (Tanaka, 1986, op cit. and references therein). Major geological map identities include the southern highlands, impact basins and plains, the northern plains, extensive volcanic and tectonic terrains, channel systems, (e.g., Valles Marineris), and the polar regions (Werner, 2010, op cit). The four basic units Pre-Noachian (oldest), Noachian, Hesperian, and Amazonian (that includes Recent sediment and ice) have the time-stratigraphic rank of System, and the chronostratigraphic rank of Period (e.g., Tanaka and Hartmann, 2012, op cit).
There have been several iterations of this framework and the chronostratigraphy (mostly at the Epoch level – Lower, Middle Upper etc.), but the overall stratigraphic scheme has remained intact. There has also been some calibration of the Martian chronology function from radiometric dating of ‘Martian meteorites’ (those that have landed on Earth), but no direct dating of in-situ rocks.
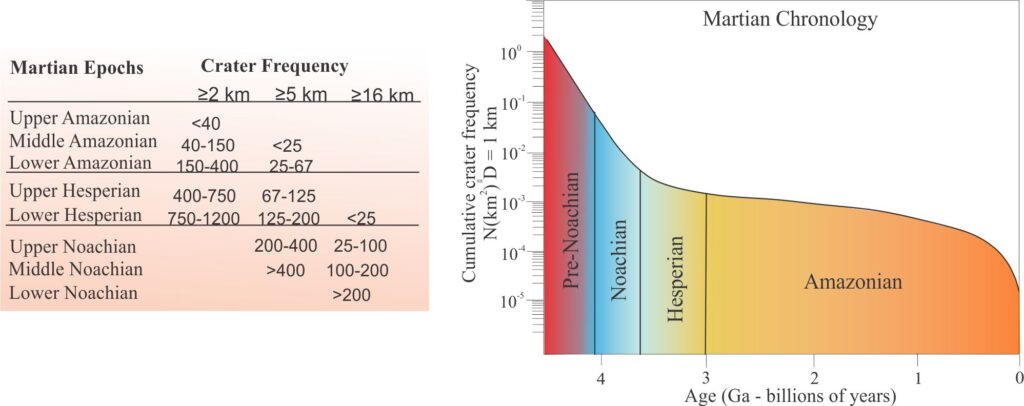
The crater counting method remains an important foundation for Martian stratigraphy. However, there are caveats because of some fundamental differences between Mars and Earth’s Moon:
- A stronger gravity field – this may influence crater depth and preservation.
- An atmosphere that could have been denser during Mars’ early history – smaller meteorites would burn up.
- Widespread volcanic activity.
- A highly skewed distribution of craters between Mars’ northern and southern hemispheres. Satellite images from Mariner 9 revealed an interesting dichotomy of two halves in Mars surface morphology: a heavily cratered southern highlands region, and a sparsely cratered northern hemisphere. Most of the southern hemisphere craters appear to have formed during the early period of heavy bombardment. It is highly unlikely that asteroid bombardment was confined to the southern hemisphere. Therefore, the difference is logically attributed to processes that resurfaced the northern craters.
- Resurfacing that completely removed or buried craters. Varying degrees of burial and degradation will influence the level of confidence in identifying and measuring crater diameters.
Crater resurfacing
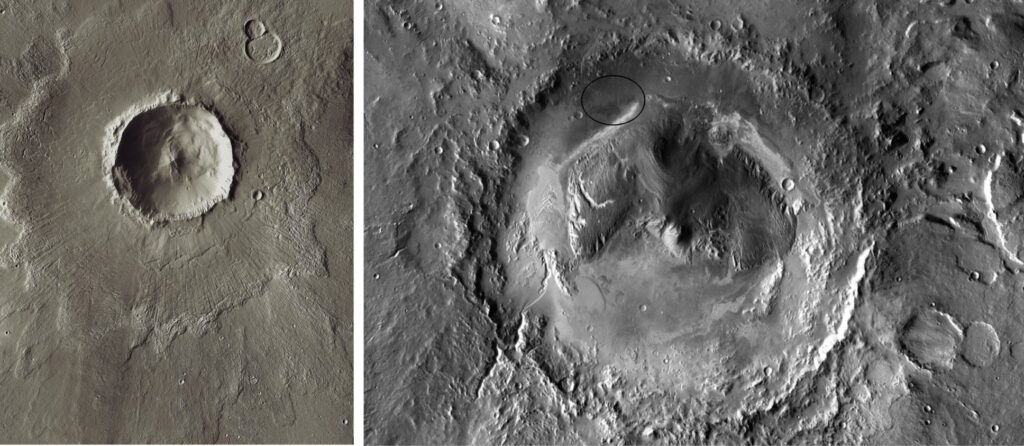
Right: Gale crater in Elysium Planitia – Southern Highlands. Its model age is 3.6 – 4.1 Ga (Noachian to Early Hesperian. Diameter is 154 km; The crater rim is highly degraded by erosion and gravitational collapse. The central peak is Mt. Sharp (Aeolis Mons). During the Noachian lacustrine deposits onlapped Mt. Sharp during rising water levels. Subsequent base-level fall eroded some of these strata, redepositing them in deeper parts of the crater basin (e.g., Heydari et al., 2023). Up to 5 km of stratigraphy on Mt Sharp presumably overlies the crater central peak bedrock. The
landing site of Curiosity Rover is indicated by the ellipse. Image Credit: NASA/JPL-Caltech/ASU
The term resurfacing refers to processes that alter and renew a planet’s surface morphology and the rocks beneath. They mostly involve weathering (when atmospheres are present), tectonic, and volcanic processes. Rocky planets like Earth, Mars, and Venus have atmospheres and hence are subjected to atmospherically generated weathering such as mass wasting, the creation and movement of sediment, chemically induced changes of bedrock and sediment, and at least on Earth biologically mediated changes. Resurfacing of Earth’s surface and crust is fundamentally driven by plate tectonics. Resurfacing of Venus’ is so advanced that barely 1000 craters have been identified across the entire surface. Mercury on the other hand is more like the Moon – much of its surface is saturated with craters (atmospheric pressures on Mercury are minuscule).
The northern hemisphere of Mars appears to be underlain by ancient, stratified sediment and lava flows. Modern aeolian processes and mass wasting continue to reshape the Martian landscape. All these processes have resulted in burial or removal of earlier-formed craters:
- Past processes involving liquid water flow in lakes, seas, rivers, and catastrophic floods.
- Volcanism – effusive (lava flows) and explosive products.
- Crater rims eroded – craters filled by sediment, lava flows.
- Mass wasting of craters by groundwater piping leading to rim collapse.
- Glaciers (water ice and/or CO2).
- Modern aeolian processes.
Mars stratigraphy and geological history
The early, post-accretion history of Mars, like the other rocky planets and moons was dominated by heavy asteroid and comet bombardment and the initiation of differentiation of a molten core from more solid crust. The oldest meteorite known (ALH 84001) is 4.091 Ga: it is an orthopyroxenite that probably represents early, mafic, magmatic differentiation and volcanism. The meteorite also contains carbonates that based on isotropic evidence indicate precipitation from relatively low temperature water (Halevy et al., 2011, OA) (earlier suggestions of microbial fossils have been discounted). One meteorite, NWA 7034 is a breccia that contains some matrix components that formed as early as 4.44 Ga, also contains significant amounts of water (Nyquist et al., 2016, OA). This data supports the hypothesis that the early Martian atmosphere contained water and CO2.
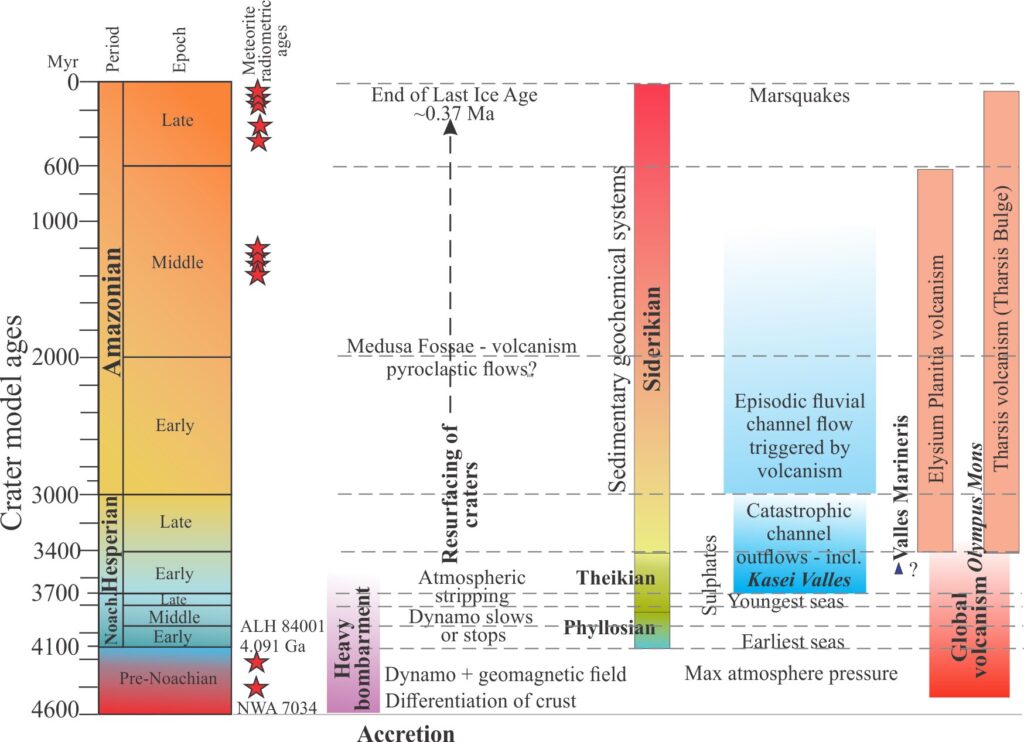
There is now abundant spectroscopic, sedimentologic, and geochemical evidence for a Martian hydrosphere during the Noachian – probably in the form of seas-oceans, lakes, fluvial systems, and groundwater. If plate tectonics played any role in Mars history, it was probably over by the end of the Noachian – hence the term ocean does not have the same connotations as it does on Earth.
However, this period of watery diversity was relatively short lived; probably less than a billion years. From the Early Hesperian and on there has been a general trend towards loss of water as liquid and vapour, along with other components of the Martian atmosphere. This prolonged, atmospheric stripping is probably related to the weakening and eventual stalling of Mars inner core dynamo and, as a consequence, its geomagnetic field. Spectroscopic data indicate that some water has survived, preserved in the polar ice caps and as permafrost.
There were more than 200 paleolakes on Noachian and Hesperian Mars. Several very long (100s of km) and deep channels or canyons (to 2.5 km deep) appear to have formed by catastrophic flooding, possibly as outbursts from these lakes (Goudge et al., 2018). Other flood hypotheses invoke melting of cryogenic groundwater (permafrost) and polar ice, and highly fluid lava flows (Gallagher and Bahia, 2021). One of the most prominent of these is Kasei Valles that is 1580 km long, up to 450 km wide, and as deep as 3 km (e.g., Robinson and Tanaka, 1990).
The final three billion years of Mars history (65%), the Amazonian Period, witnessed the continued stripping of its atmosphere, and surface geochemistry dominated by anhydrous oxidation of iron-bearing minerals – the so called Siderikian (an informal geochemical period). Fluvial activity became increasingly episodic, possibly initiated by cryogenic melts during volcanic activity, particularly in the extensive Elysium Planitia and Tharsis volcanic fields. Some meteorite ages indicate active volcanism as recent as1-2 million years ago. Aeolian processes became widespread during the Amazonian, manifested as extensive dune fields. Resurfacing of craters continued with sand infills, mass wasting that in some cases is triggered by groundwater seepage or erosion by glaciers.
There are even a few Anthropocene trace fossils – from lander impacts and rover tire tracks.
The same three billion years on Earth witnessed the maturing of plate tectonics and flourishing life forms interrupted by the occasional extinction event, some of which were triggered by bolide impacts.