
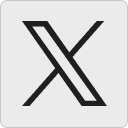
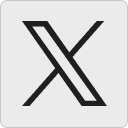
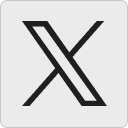










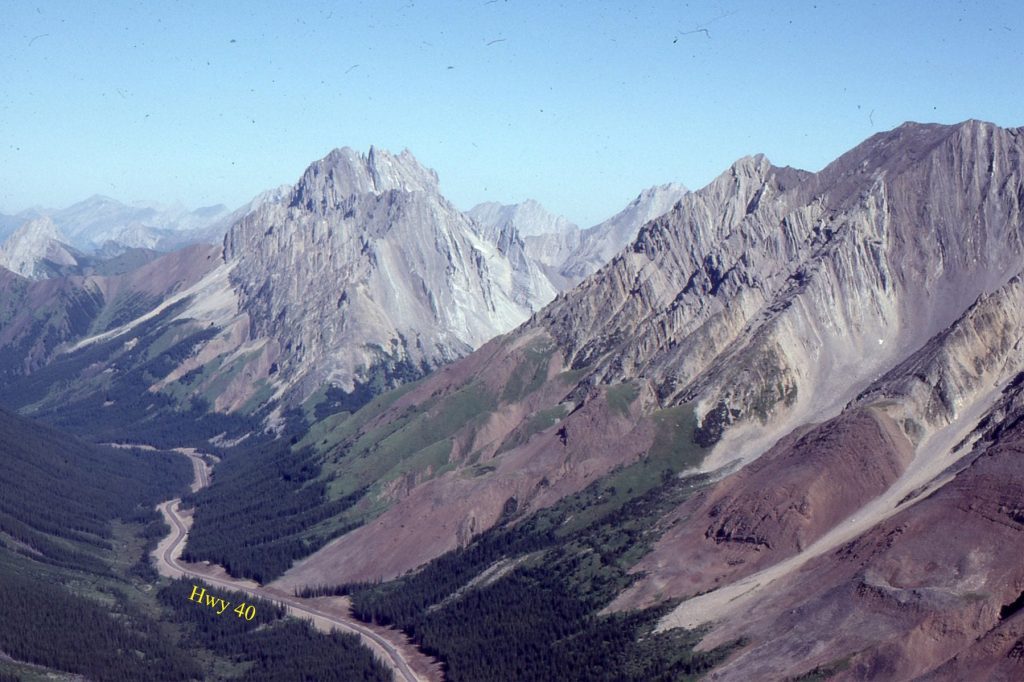
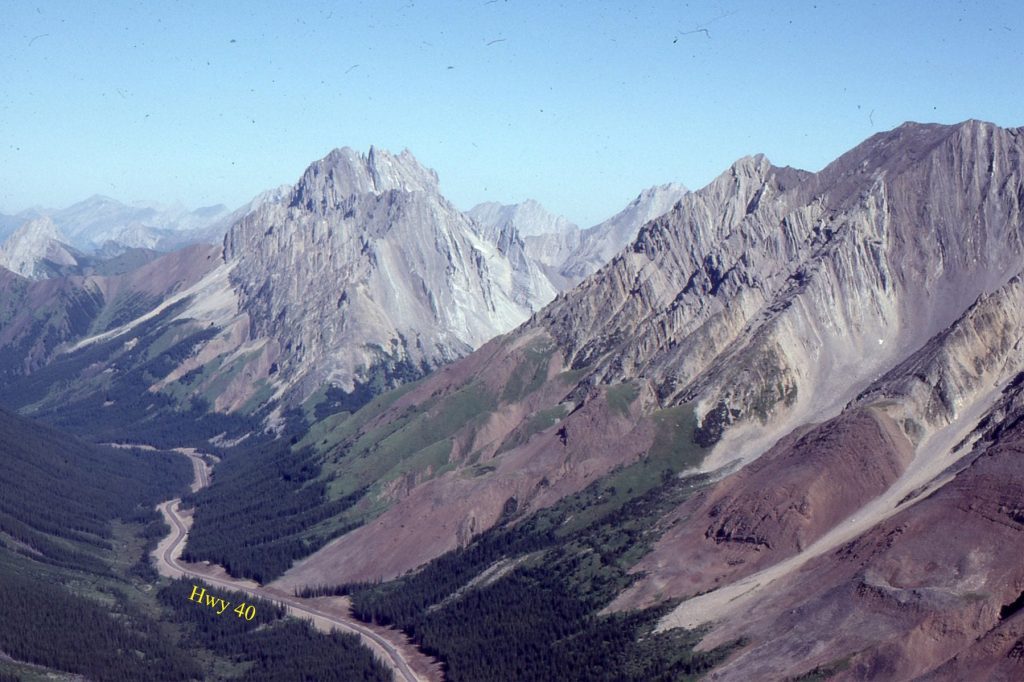
Part of the Alberta Front Ranges fold -thrust belt north of Highwood Pass. Sawtooth-like Lower Paleozoic carbonates are exposed as flatirons in the hanging wall of a thrust immediately east of Lewis Thrust. Late Jurassic – Early Cretaceous foredeep deposits in the valley floor (mostly covered) were involved in the deformation as the orogenic load migrated eastward. Orogenic transport to the right.
Basins formed by flexure of oceanic and continental lithosphere; foreland basin exemplars.
What is flexure?
The way we define the rheological behaviour of tectonic plates creates an interesting paradox. We consider plates to be rigid, and yet we allow them to be non-rigid. Under certain conditions they behave elastically, under other conditions in a viscous or ductile manner. Lithospheric flexure is one of these non-rigid responses; the lithosphere can bend under applied loads and recover elastically if the load is removed. We are witness to this recovery in Scandinavia and Canada where there is measurable uplift or rebound on the heal of the Last Glaciation.
Lithospheric flexure also depends on a reaction in the underlying mantle. Dynamic topography, at wavelengths of several 100 km, is a manifestation of mantle convection. The development of oceanic trenches at subduction zones is partly a function of convection-induced subsidence. Likewise, foreland basin subsidence is probably caused by a combination of tectonic (orogenic) supracrustal loading and dynamic mantle loading by downwelling convection cells.
The oceanic lithosphere is a good place to start examining the causes and consequences of flexure, because it is relatively simple from a compositional and rheological perspective (compared with continental lithosphere). Lithospheric flexure occurs in subduction zones where the oceanographic expression is deep, long, linear trenches. Flexure also occurs beneath large volcanic islands like Hawaii, in this case producing a moat around the islands. In both cases, flexure occurs over wavelengths of 200-300 km.
Lithospheric flexure can be described as a sinusoidal wave. The bathymetric expression of this wave in subduction zones and oceanic islands reveals three main morphological and structural components: the load, a basin that forms immediately outboard of the load, and a forebulge (or peripheral bulge); the forebulge is the mechanical response to flexure of an elastic plate. The amplitude of the forebulge is a small fraction of the total flexure beneath the load. These components are also common to foreland basins on continental lithosphere.
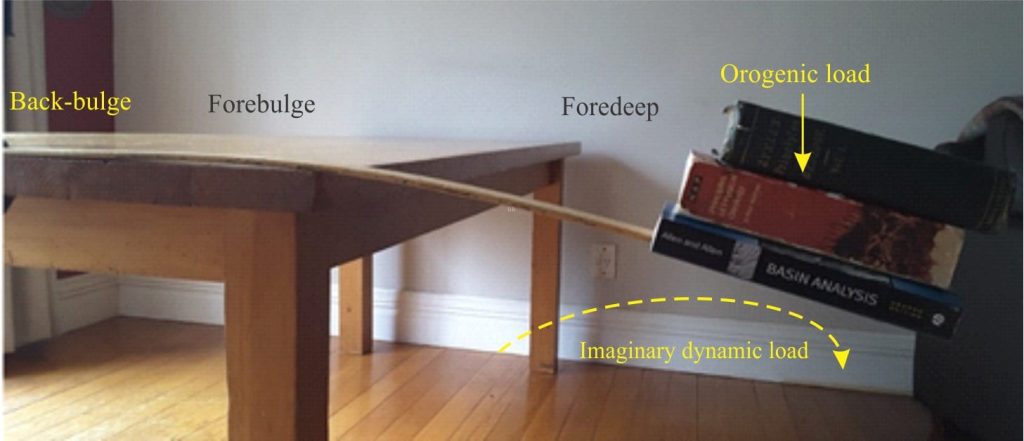
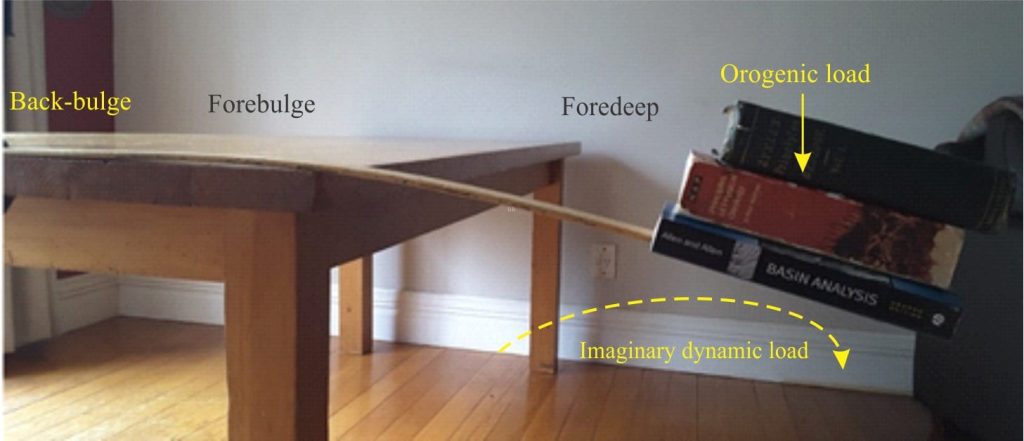
Flexure of a thin, continuous beam beneath a deforming orogen (and an imaginary mantle dynamic load). The amplitude of the forebulge is a small fraction of total flexure.
Note that loads can act:
- Vertically as is the case for a volcanic edifice or stack of thrust sheets,
- As horizontally applied stresses such as mid-ocean ridge push,
- As a torque that results in bending, and
- In the mantle by dynamic loading beneath the flexing plate.
Vertical loading by volcanic islands is relatively straight forward. Loading of a subducting slab is more complicated because it involves horizontal push stresses (from a spreading ridge), vertical loading of dense oceanic lithosphere as it sinks, and bending loads where the lithosphere is deflected downwards.
Flexure of oceanic crust depends not just on the load, but on two other factors:
- The age of the crust; older crust is colder, thicker, and mechanically stronger, and
- The elastic thickness of the lithosphere; thicker lithosphere will flex less than thinner lithosphere.


Left: A bathymetric profile (NE-SW) across the trend of the Emperor volcanic chain, between Oahu and Molokai, shows clear differentiation of the flexural moat and forebulge (on either side of the volcanic chain). Right: Modelled flexural curves for different elastic thicknesses. The Te that best fits is Te = 30 km. From Watts and ten Brink, 1989, Fig 1, Fig. 23.
For the Hawaiian example, a north-south bathymetric profile offshore between Oahu and Molokai clearly shows the moat (basin) and forebulge; the amplitude of the bulge is about 200-400 m, and its wavelength between 100-150 km. The modelled flexural profile (Watts and ten Brink, 1989) is oriented along the axis of the island chain from Molokai to Hawaii. It shows significant flexure towards the massive volcanic load imposed by the big island Hawaii where the volcanic edifice is more than 10km high. The best fitting modelled thickness of the elastic lithosphere is 30 km (Zhong and Watts, 2013, open access) which indicates that the lithosphere has sagged about 4.5 km beneath the load.
Foreland basins
Foreland basins are the flexural response to tectonic loading of continental lithosphere. Like oceanic trenches they are elongate parallel to the load. However, they are fundamentally different to oceanic basins in several respects (information mainly from DeCelles (2012 – a nice review, PDF available); Allen and Allen, 2013):
- The basins form over continental crust that is compositionally more felsic, has greater thickness, and is rheologically more complex than oceanic crust. This complexity is exacerbated by internal structural and compositional discontinuities acquired over, in many cases, a billion years and more, including several episodes of mountain building and igneous intrusion.
- Foreland basins occur in upper and lower plates depending on the setting of plate convergence. For Andean-type orogens (oceanic trench – continent convergent boundaries) the retroarc foreland basin is in the upper plate (the trench is on the lower, subducting plate). Continent-continent type collisional margins may contain basins on both the lower plate (peripheral foreland basins) and upper plate (retro-foreland basins).
- Loading and flexure are dynamically linked to an evolving orogenic belt. The orogen itself forms under compression, where stacking of thrust sheets results in shortening of the upper crust by 100s of kilometres. It is this telescoping of thrust sheets that creates a dynamic supracrustal load – dynamic in the sense that each thrust event increases the topographic load, and therefore the amount of flexural subsidence.
- Dynamic loading coupled to mantle convection may also contribute to subsidence. In this case, the wavelength of any dynamic topographic effects means that the entire foreland basin will be affected.
- Development of topography is accompanied by evolving orographic effects on the distribution of precipitation, erosion, and delivery of sediment to the basin.
- The amount of flexural subsidence in a foreland basin depends on the load, the elastic thickness of the lithosphere, and whether the flexing plate is continuous or broken; a broken plate is one with significant structural discontinuities, such as terrane or old plate boundaries. Flexure is fundamentally an elastic (or possibly viscoelastic) response. An important corollary of this behaviour is that the lithosphere will also respond to unloading. Erosion of elevated terrain accomplishes two things: it reduces the load, and it provides sediment for the adjacent foreland basin. Erosion, if it proceeds far enough, will induce an isostatic response – namely, uplift (e.g. Heller et al. 1988). The change from thrust load induced flexural subsidence to isostatically induced uplift may be preserved in the basin stratigraphic record as an influx of conglomerate.
Thin interbedded fluvial sandstone-mudstone (uppermost Elk Formation, Lower Cretaceous) abruptly overlain by crossbedded conglomerate (braided river facies – Cadomin Formation). The gravel pulse was probably shed across the wedge top during uplift and erosion of the Early Cretaceous orogenic topography.
- Foreland basins have asymmetric profiles, deepest close to the frontal thrusts of the orogen.
- A useful 4-fold partitioning of foreland basins (DeCelles and Giles, 1996), based on structural position and the loci of deposition, recognises a (structural) wedge top depozone, a foredeep outboard of the frontal thrust, a forebulge that is part of the flexural wave, and a shallow back-bulge area that tapers toward the continent to a basin margin.
- The basin axis will migrate towards the continent in concert with the flexural wave during incremental orogenic loading. The foredeep, forebulge and back-bulge will move in tandem with these axial excursions. This is illustrated below for the Western Interior Basin over the period Campanian to Paleocene – in this case the hingeline between foredeep and forebulge is plotted. There is a general northward migration of the foredeep over about 25 million years, albeit with a few twists and turns. The driving force for this pulse of foreland basin development corresponds with the docking of Insular superterrane to the western margin of North America (Insular Superterrane consists primarily of Wrangellia and Alexander terranes.
Fore deep migration mapped for a 25 million year interval (Campanian to Early Paleocene), for the Western Interior (foreland) Basin. EC = Early Campanian; MC = middle Campanian; LC = Late Campanian; EM = Early Maastrichtian; EP = Early Paleocene. From Miall and Catuneanu, 2019.
- Foreland basins are filled with sediment derived from the adjacent orogen. Stratigraphic thicknesses commonly exceed 5 km.
- Proximal foredeep deposits inevitably become involved in the deformation as the frontal thrust moves towards the continent.
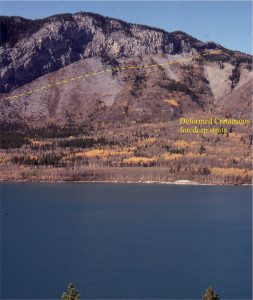
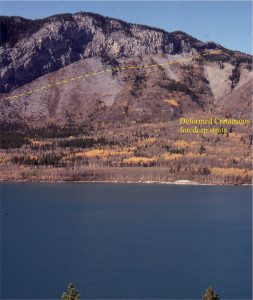
Devonian-Mississippian carbonates thrust over softer, less resistant Upper Cretaceous foredeep deposits.
A few notes on foreland basin depozones
Each depozone is characterized, in a general way, by sedimentary processes and stratigraphic architecture that reflect proximity to the topographic load, periodically active thrust faults and associated antithetic-synthetic structures, and depozone migration in concert with the flexural wave. Thus, in an ideal case, the wedge top will eventually overlie and perhaps structurally incorporate deposits of the axial foredeep, that in turn will overlie deposits on the peripheral bulge and back-bulge. These transitions may be represented stratigraphically, with wedge top deposits at the top of the pile. However, given the structural dynamics of these basins, deposits from any of the depozones may be missing, or cannibalised by the advancing thrust front.
Wedge top
The surface created at the front of an orogenic wedge is often topographically low relative to thrust-related topography in the older part of the orogen. The locus of deposition across the wedge top will to a large extent be guided by faulting; deposits may even be carried piggy-back style with advancing frontal thrusts. Deposits will typically form coarse-grained alluvial fans and low-sinuosity fluvial channels that eventually merge with the adjacent foredeep. Finer grained sediment will mostly bypass the wedge top and accumulate in the foredeep. Basinward migration of the orogenic wedge may see older foredeep and wedge top deposits cannibalised and reworked into younger segments of the wedge top. Structurally induced unconformities are common.
The foredeep
Foredeeps are the most extensive, deepest, and stratigraphically the thickest depozone of any foreland basin. Although coarse-grained sediment may spill across the shallow, foredeep coast, the bulk of sediment in this depozone is dominated by sand and mud. The foredeep axis is closest to the deformation front and thus is the deepest part of the basin, shallowing towards the peripheral bulge.
Fluvial depositional systems, originating on the wedge top, may feed deltas or shallow shelves; these systems can develop on both the orogen and forebulge margins. If marine, these shallow environments will be subjected to tectonically and eustatically induced sea level fluctuations where a shelf is exposed to fluvial processes, and where sediment is transported to the deep basin to accumulate as submarine fans.
The forebulge
With theoretical amplitudes on the order of 10s of metres, and widths <100 km to >400 km, any forebulge will be a relative subtle topographic feature compared to the adjacent foredeep. In real foreland basins the stratigraphic record of forebulge development is commonly ambiguous.
Sediment supply is mainly from the foredeep with contributions from the continent and back-bulge basin. Carbonate platforms may develop where the supply of terrigenous sediment is low. Where submerged they are represented by thin sedimentary units including condensed stratigraphic sections. Where exposed they are subjected to erosion and development of regoliths, and in some cases aeolian deposition. Unconformities are common. The principal stratigraphic architectures involve onlap on the orogen side, and downlap on the continent side of the bulge.
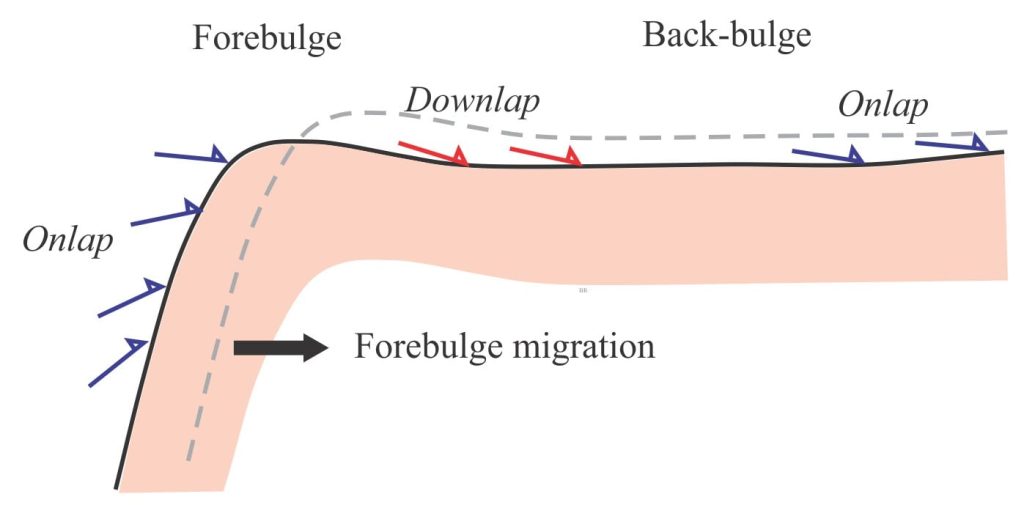
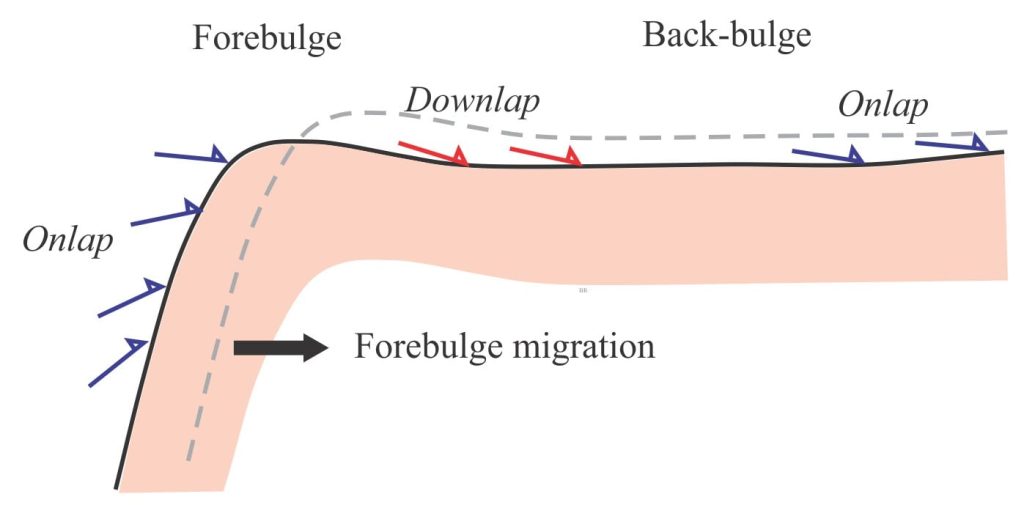
Typical lapout relationships of stratigraphic units across the forebulge and back-bulge depozones. Changes in the location of the bulge with the flexural wave will result in unconformities across most stratigraphic surfaces. From DeCelles, 2012, Fig. 20.1
Back-bulge
The depozone between the forebulge and continent is shallow; sediment is derived from the foredeep but tends to be fine-grained. Some sediment will be contributed by the exposed continent. Strata will onlap the unconformity with continental rocks.
Other posts in this series
Sedimentary basins: Regions of prolonged subsidence
The rheology of the lithosphere
Isostasy: A lithospheric balancing act
The thermal structure of the lithosphere
Classification of sedimentary basins
Stretching the lithosphere: Rift basins
Nascent, conjugate passive margins
Thrust faults: Some common terminology
Accretionary prisms and forearc basins
Basins formed by strike-slip tectonics
Allochthonous terranes – suspect and exotic
Source to sink: Sediment routing systems
Geohistory 1: Accounting for basin subsidence
Geohistory 2: Backstripping tectonic subsidence



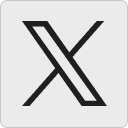
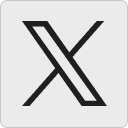
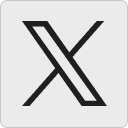













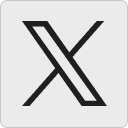
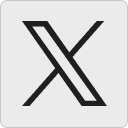
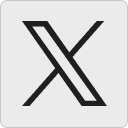


